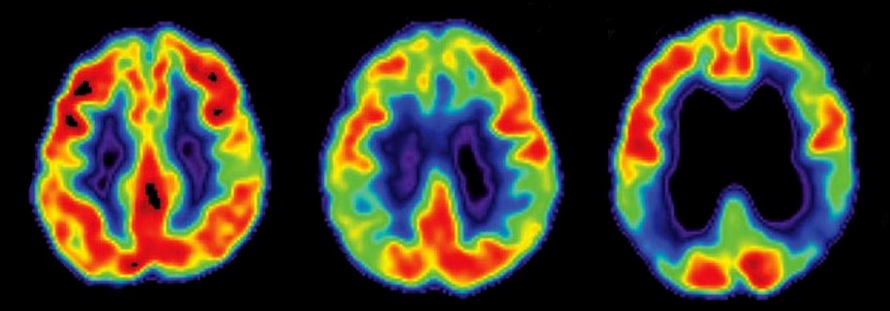
Navigation
Neurodegeneration Products Dashboard
Aβ(this page)
tau
α-synuclein
TDP-43
C9ORF72
Huntingtin
Ordering Information
Neurodegeneration-related Flyers and Brochures
Antibodies for Neurodegeneration Research (TDP-43, 4R-tau, C9ORF, Synuclein)
PDF Download
Oxidative Stress Markers
mAbs and Selection Guide
PDF Download
Autophagy/Mitophagy Antibodies
PDF Download
Glutamate and NMDA related
Dopamine related
Cholesterol
Ganglioside
Glucosylceramide
Sphingosine
Mitochondria related
Heat shock
Oxidative stress
DNA damage
Lipid peroxidation
Protein carbonylation
Senescence
Transcription and Chromatin
Autophagy
Nucleocytoplasmic transport
Prion protein
APOETREM2
Progranulin
SOD1
FUS
Humanin
Gfap
Paraoxonase
CD36
Dysbindin
BDNF
VEGF
PARP
PPAR gamma
Ubiquitin
Proteasome
Proteases
Caspases
Peptidyl-prolyl cis-trans isomerases
NADPH oxidases
Dyneins
Kinesins
Kinases and Kinase inhibitors
Signaling molecules
Nuclear Hormone Receptors
Products for Neurodegeneration Research: Aβ
Catalog Number | Product | Reactivity | Unit size |
CAC-YCU-MK-AP01 | Anti Amyloid-Beta Precursor Protein (APP) mAb (Clone 278) | Human | 100UG |
CAC-YCU-MK-BA01 | Anti Amyloid-Beta Precursor Protein (APP) mAb (Clone 59) | Human | 100UG |
BAM-74-104-EX | Anti Amyloid-Beta Precursor Protein (APP) C-terminal region pAb (Rabbit, Antiserum) | Human, Rat, Mouse | 100UL |
BAM-74-106-EX | Anti Amyloid-Beta Precursor Protein (APP) N-terminal region pAb (Rabbit, Antiserum) | Human, Rat, Mouse | 100UL |
BAM-74-108-EX | Anti Amyloid-Beta Precursor Protein-695 C-terminal 31 residue fragment (APP695-C31) pAb (Rabbit, Antiserum) | Human, Rat, Mouse | 100UL |
BAM-74-110-EX | Anti Amyloid-Beta Precursor Protein-695 N-terminal ΔC31 fragment (APP695-ΔC31) pAb (Rabbit, Antiserum) | Human, Rat, Mouse | 100UL |
BDX-4BDX-1503S | Anti-APP [pT668] pAb | Human, Rat, Mouse | 40UL and 80UL |
YII-YA010-EX | Anti Amyloid Beta Precursor Protein (APP) Residues 1-40 pAb (Rabbit, Antiserum) | Human | 50UL |
CSR-KN-TOYU-M03 | Amyloid Beta Peptide 40 | Mammal | 1MG |
CSR-KN-TOYU-M04 | Amyloid Beta Peptide 42 | Mammal | 1MG |
CSR-SYN02 | Amyloid Fluorescent Staining Kit | - | 100 tests |
PRX-MKA1149 | Anti BACE1 pAb (Rabbit, Antiserum) | Mouse | 100UL |
PRX-MKA1149PA | Anti BACE1 pAb (Rabbit, Purified Ig) | Mouse | 100UG |
KAL-KR078 | Anti Aph-1 Homolog A, Gamma-Secretase Subunit (APH1A) pAb (Rabbit, Affinity Purified) | Human | 25UG |
CAC-ACC-PA001 | Anti Parvalbumin Alpha pAb (Rabbit, Affinity Purified) | Human, Rat | 50UG |
Alzheimer’s disease (AD) is a progressive neurodegenerative disorder characterized by memory impairment and cognitive decline that can ultimately affect behavior, speech, visuospatial orientation and the motor system. The leading cause of dementia worldwide, AD is associated with synaptic loss, neuronal degeneration and hippocampal and cortical atrophy; at death, the brain of an AD patient may weigh a third less than that of an age-matched control. The cardinal histopathological features of AD, first described more than 100 years ago by Alois Alzheimer, are nonvascular extracellular senile plaques and intraneuronal neurofibrillary tangles (NFTs). In the mid-1980s plaques and tangles were discovered to comprise, respectively, fibrillary Aβ (amyloid-beta) peptides and hyperphosphorylated tau protein aggregates. Hope that these two molecular entities would leverage a long sought clinical breakthrough released a torrent of investigation into their potential pathomechanistic roles in AD. Please scroll to the ORDERING section to explore CosmoBio USA's Aβ reagents that have proven helpful in the study of neurodegenerative diseases.
From: Chávez-Gutiérrez, L., Szaruga, M. (2020). Mechanisms of neurodegeneration — Insights from familial Alzheimer’s disease Seminars in Cell & Developmental Biology.
Most AD cases (95-99%) are late onset and sporadic (SAD). The major risk factor for SAD is age, with the incidence for all dementias doubling every 6.3 years from ~4 per 1000 for ages 60-90 to ~105 per 1000 above age 90. Prevalence is estimated at 10% for individuals over 65 years and 40% for those over 80 years. There is also a strong genetic contribution to SAD. The list of disease risk loci identified by GWAS currently numbers over 30, with variants of APOE and TREM2 conferring the highest risks. Inheritance of one copy of the APOEε4 allele increases AD risk by ~3-fold while two copies increases it by ~12-fold. Compared to APOEε4-negative AD patients, those who carry at least one APOEε4 allele exhibit earlier disease onset, faster disease progression and increased brain atrophy. Inheritance of the most common TREM2 variant (R47H), that exhibits impaired Aβ binding, increases the risk of developing AD by ~4-fold.
In the 1990s and early 2000s, study of a rare, early onset (< age 65), autosomal dominant, familial form of AD (FAD) led to the discovery of FAD-linked mutations clustered within three different genes: APP, PSEN1 and PSEN2, respectively, accounting for ~14%, ~81% and ~6% of FAD cases. The chromosome 21 APP gene encodes the evolutionarily ancient, amyloid precursor protein, a 695 amino acid transmembrane polypeptide required for neuronal development, neurite outgrowth, axonal transport and synaptic function. APP undergoes complex proteolytic processing along distinct major and minor pathways into multiple sub fragments by the coordinated activity of three endopeptidases, referred to as α-secretase, β-secretase and γ-secretase. In the dominant non-amyloidogenic pathway APP is cleaved by α-secretase at a position 83 amino acids from the C terminus (in the Aβ region), liberating a large N-terminal ecto-fragment called sAPPα. The remaining 83-amino acid C-terminal fragment (C83) is retained in the membrane and subsequently cleaved by γ-secretase, producing short N- and C-terminal fragments termed, respectively, p3 and AICD. The minor amyloidogenic APP cleavage pathway involves β-secretase rather than α-secretase. In this pathway, β-secretase makes the initial cut at a position located 99 amino acids from the APP C terminus, resulting in the liberation of an N-terminal ecto-fragment (sAPPβ) 16 residues smaller than sAPPα. Left in the membrane is a 99 amino acid C-terminal fragment (C99) with a newly exposed N terminus that corresponds to the first amino acid of Aβ. Subsequent cleavage of C99 (between residues 38 and 43) by γ-secretase liberates variably sized Aβ peptides. While most Aβ peptides are 40 residues in length (Aβ40), ~10% are a 42-residue variant (Aβ42). Aβ42 is more hydrophobic and hence more prone to aggregation into amyloid fibrils than Aβ40, and it is this longer form that is predominant in senile plaques.
PSEN1 and PSEN2 encode alternate versions of the presenilin protein that, together with nicastrin (NCSTN), PSEN enhancer (PEN2) and anterior pharynx 1 (either APH1a or APH1b), comprise the tetrameric γ-secretase complex. Most FAD mutations either elevate Aβ peptide production rate or destabilize the γ-secretase-APP interaction, leading to generation of longer and more aggregation-prone Aβ peptides, like Aβ42. Of note, complete loss-of-function mutations in PSEN (as well as in other components of the γ-secretase complex) cause the chronic skin disease acne inversa and not AD, indicating the importance of gain-of-function mechanisms for FAD pathogenesis.
Aβ, initially considered a homogeneous entity, is now understood to exist in vivo in distinct structural and functional states: (1) physiological, soluble monomers, (2) pathological, soluble, misfolded oligomers, and (3) pathological, insoluble, fibrillar aggregates. Generated in synaptic processes and homeostatically maintained at relatively high production and turnover rates, monomeric Aβ modulates synaptic activity and is thought to play a role in synaptic vesicle cycling. From a physicochemical perspective, the Aβ monomer belongs to the class of intrinsically disordered proteins (IDP) that lack a fixed three-dimensional structure. Monomeric Aβ is metastable in aqueous solutions and gains stable secondary structure in membrane-like environments. Although monomeric Aβ (in particular, Aβ42) is prone to aggregation, chaperone-mediated folding control likely ensures orderly biosynthesis and turnover in non-diseased young and older individuals. Importantly, Aβ monomers can undergo a structural transformation into a misfolded, detergent-soluble, oligomeric form that comprises ~1% of intracellular Aβ and accounts for most of its toxicity. Whereas Aβ oligomers recovered from AD brains dissolve in SDS-containing buffers, dissolution of fibrillar Aβ requires low pH as can be achieved in formic acid. Interestingly, Aβ fibrils adopt a well-defined and stable structure that grows by the addition of Aβ monomers but not Aβ oligomers, indicating that oligomers are not direct intermediates on the fibril folding pathway.
In tissue derived from human brain, toxic Aβ oligomerization initiates within cells rather than in the extracellular space. Work from animal models supports this view. Two different oligomer-specific antibodies showed that in 3xTg-AD mice Aβ oligomerization begins intracellularly. Other studies revealed that Aβ oligomerization occurs in the endosomal compartment during interactions with lipid bilayers, especially in lipid raft microdomains rich in cholesterol and glycosphingolipid. Aβ oligomerization is accelerated through interaction with clusters of monosialogangliosides in lipid rafts where Aβ bound to GM1 ganglioside can nucleate further Aβ aggregation. GM1 levels in lipid rafts of synaptosomes have been shown to increase with age, consistent with progressive increase in Aβ aggregates with age. Together, these results suggest that lipid rafts containing ganglioside clusters can serve as conformational catalysts or chaperones to generate seeding-competent, membrane-bound forms of Aβ, and that plasma and intracellular membranes are vital for the generation of oligomeric Aβ species.
In transgenic mouse models the most pathological Aβ species are oligomers. At 4 months of age when profound deficits in long-term potentiation (LTP) are first detected in the 3xTg-AD mouse model of AD, there is abundant intraneuronal Aβ accumulation but no plaque formation, little somatodendritic tau and no hyperphosphorylated tau. Removal of intraneuronal Aβ with immunotherapy restores cognition to control non-transgenic levels. A recent study found that Aβ oligomers induced LTP deficits in hippocampal slice cultures, and that these deficits could be rescued by increased UCHL1 (ubiquitin C-terminal hydrolase L1). UCHL1 activity is diminished in 3xTg-AD mice at time points corresponding with LTP deficits and intraneuronal accumulation of Aβ oligomers. Further, this hydrolase is an integral component of the ubiquitin-proteasome system, which has been found to be impaired as a result of the accumulation of Aβ within multivesicular bodies (MVBs) in neurons. Proteasome inhibition, both in vivo and in vitro, leads to higher Aβ levels, suggesting that the proteasome contributes to intracellular Aβ degradation. Aβ accumulation has also been observed in the mitochondria of Tg2576 mice. Moreover, progressive mitochondrial Aβ accumulation is associated with diminished enzymatic activity of respiratory chain complexes III and IV and a reduced rate of oxygen consumption. These observations may help to explain the mitochondrial defects described in AD and mouse models of the disease.
Despite the established toxicity of oligomeric Aβ, it has long been known that NFTs rather than senile plaques correlate best with neuronal cell loss and cognitive decline. Newly developed Aβ- and tau-specific PET tracers have revealed that many normal individuals harbor significant amyloid deposits while some AD patients possess very few. Additionally, in the brains of elderly non-demented patients, the distribution of senile plaques was sometimes as extensive as that in dementia patients. By contrast, tau deposition correlated very well with the onset and specific nature of cognitive decline and with the neuro-anatomical locus of neurodegneration.
Tau is a microtubule-associated protein that plays an important role in microtubule assembly and stabilization. Human tau is encoded by the microtubule-associated protein tau (MAPT) gene which comprises 16 exons on chromosome 17q21. In the adult human brain, tau is mainly found in neurons where it exists in six different isoforms generated by alternative splicing of exon 2 (E2), E3 and E10. Isoforms that contain zero, one or two N-terminal 29-residue inserts (encoded by both E2 and E3) are known as 0N, 1N and 2N, respectively. Isoforms also differ in their inclusion of E10 which encodes one of four C-terminal repeat domains resulting in isoforms with 3 or 4 repeats, called 3R and 4R, respectively. The alternative splicing of E10 is of particular interest, as it is associated with distinct tauopathies that can be classified into three groups based on the tau isoforms found in NFTs: 4R tauopathies (including PSP, CBD and AGD), 3R tauopathies (for example, PiD) and 3R+4R tauopathies in AD.
Compared to 3R tau, the additional repeat domain in 4R tau confers higher affinity for microtubules and greater efficiency at promoting microtubule assembly. However, the function of the two N-terminal inserts remains unclear. Comprising part of the projection domain of tau, N-terminal inserts may influence the attachment or spacing between microtubules and other cell components. They may also influence the subcellular distribution of tau. In mice, 0N, 1N and 2N tau isoforms each show distinct subcellular compartmentalization. Unlike the human brain, which expresses all six tau isoforms, the adult mouse brain almost exclusively expresses the three 4R tau isoforms (0N4R, 1N4R and 2N4R), while 3R tau is only transiently expressed in mouse newborn neurons. Mouse and human tau proteins are highly homologous between N1 and the C terminus (with 92% sequence similarity) but differ considerably over the N-terminal header (with just 57% sequence similarity).
Based on microtubule interactions and amino acid character, tau comprises two major domains (N-terminal projection domain and C-terminal assembly domain) separated by a Pro-rich region containing seven PXXP motifs that serve as binding sites for signaling proteins with SH3 domains, such as the tyrosine kinase FYN. Although tau is the main component of paired helical filaments (PHFs) found in AD and other tauopathies, NMR and small-angle X-ray scattering show that physiological tau is ‘natively unfolded’ or ‘intrinsically disordered’ and has little tendency for aggregation. However, tau may form local conformations when it binds to other interacting proteins and partners (such as microtubules). In addition, despite its unfolded character, the tau molecule shows a preference for adopting a paperclip-like global conformation, in which the N-terminal, C-terminal and repeat domains all approach each other. Formation of the paperclip structure might protect tau from aggregation. Conversely, truncation of tau, which prevents formation of this structure, might thereby promote its aggregation.
The processes at the level of mRNA and/or protein underlying the polarized distribution of tau in adult neurons remain poorly understood. An axon localization signal in the 3ʹ untranslated region tends to distribute Tau mRNA towards proximal axons. A 5ʹ terminal oligopyrimidine (5ʹ TOP) tract, which mediates mTOR-governed protein synthesis, promotes preferential translation of tau mRNA in axons. At the protein level, several factors may contribute to the axonal localization of tau, including faster turn-over of tau in the somatodendritic compartment than in axons; higher affinity of tau for microtubules in axons than in dendrites; fast axonal transport of tau soon after its synthesis in the cell body; and an axon initial segment barrier against retrograde diffusion of tau into dendrites. As mentioned above, subcellular sorting of tau seems to be isoform dependent. In human AD brains, the missorting of tau into dendrites represents one of the early signs of neurodegeneration. How the different sorting mechanisms are involved in tau missorting remains unclear. Given the differential distribution of tau (and tau isoforms) in different cell compartments, it is likely that the protein serves different functions in different environments. Thus, disturbance of tau sorting may induce toxic gain of function and contribute to neurodegeneration.
Phosphorylation plays a crucial part in regulating the physiological functions of tau, including its binding to microtubules, and therefore regulates the assembly and stability of microtubules themselves. For example, phosphorylation by MARK, PKA or CaMKII of KXGS motifs (particularly Ser262) in the repeat domain can reduce the affinity of tau for microtubules. In addition, phosphorylation of Ser214 and Thr231 can trigger the detachment of tau from microtubules, whereas phosphorylation at other Thr-Pro or Ser-Pro motifs in the flanking region has only a weak influence on tau-microtubule binding. Thr231 phosphorylation of tau triggers a trans-to-cis isomerization that reduces its affinity for microtubules. The peptidyl-prolyl cis–trans isomerase PIN1 can convert cis tau back to a trans conformation and thereby restore microtubule-binding activity. Cis tau has been found in the brains of humans or mice after traumatic brain injury and in individuals with mild cognitive impairment or AD.
Hyperphosphorylation of tau may induce pathology through a variety of mechanisms. First, hyperphosphorylation of tau might induce missorting from axons to somatodendritic compartments, which can cause synaptic dysfunction. Second, tau phosphorylation may alter its proteasomal or autophagic degradation and its truncation by proteases. For instance, the phosphorylation of tau at Ser422 inhibits the cleavage of tau by caspase 3 at Asp421. And tau phosphorylated at Ser262 or Ser356 cannot be recognized by the C terminus of the HSP70-interacting protein–heat shock protein 90 (CHIP-HSP90) complex and thus escapes from proteasomal degradation. Third, phosphorylation of tau is often considered to enhance tau aggregation, as hyperphosphorylation and aggregation are both increased in AD. Last, phosphorylation may change the association of tau with its interaction partners. For example, hyperphosphorylated tau but not unphosphorylated tau can interact with the kinesin-associated protein JUN N-terminal kinase-interacting protein 1 (JIP1) and thus impair the formation of the kinesin complex, which mediates axonal transport.
There are as many as 85 potential phosphorylation sites (80 Ser/Thr and 5 Tyr) in the longest tau isoform (2N4R), and most of these are accessible owing to its unfolded structure. Approximately 45 of these phosphorylation sites have been observed experimentally. Tau phosphorylation sites cluster in the flanking regions where there are 17 Thr-Pro or Ser-Pro motifs of particular interest. These motifs are abnormally hyper-phosphorylated in AD and other tauopathies and are targets of several signal-transducing proline-directed serine/threonine kinases. Other phosphorylation sites in or near the repeat domain are phosphorylated by MARKs (also known as PAR1 kinases), PKA and CaMKII, among others. In addition, tau can be phosphorylated by tyrosine kinases such as the SRC family members LCK, SYK and FYN at Tyr18, and the ABL family members ARG and ABL1 at Tyr394. PHFs in the brains of individuals with AD exhibit tau phosphorylated at Tyr18 and Tyr394. However, in this case — as in the cases of other sites — the occupancy of these sites is not 100%, as NFTs in AD also react with an antibody specific for tau that is not phosphorylated at Tyr18. In a line of the JNPL3 tauopathy mouse model, which expresses human 0N4R tau bearing the missense P301L mutation, the overall increase in Tyr phosphorylation of tau correlated with the formation of tau aggregates, suggesting that overall Tyr phosphorylation might contribute to tau aggregation.
Protein phosphatase 1 (PP1), PP2A, PP2B, PP2C and PP5 have all been implicated in the dephosphorylation of tau. Among these, PP2A is the main phosphatase, accounting for ~70% of human brain tau phosphatase activity. Importantly, PP2A activity is reduced in the AD brain by ~20% and ~40% in the grey and white matter, respectively. The reduction of PP2A activity in AD could be due to post-translational modifications of its catalytic domain, decreased PP2A expression or increased levels of the endogenous PP2A inhibitors, I1PP2A and I2PP2A. It is worth noting that compared with kinases, phosphatases are more sensitive to temperature changes, as hypothermia inhibits phosphatases exponentially, but inhibits kinase activities only linearly. This may explain why tau hyperphosphorylation occurs during animal hibernation and in anesthesia-induced hypothermia.
It appears that synaptic loss in AD precedes neuronal loss, and these effects are probably driven by amyloid and tau pathology. Numerous studies of synaptic protein markers as well as electron microscopy have documented synaptic loss in AD and amnestic mild cognitive impairment that is thought to precede AD. Synaptic loss appears to be the strongest correlate of cognitive decline in AD, surpassing the associations with neuronal loss and tau burden. Cognitive decline and decreases in verbal fluency early in AD are believed to reflect these decreases in synaptic density in the hippocampus and medial temporal lobes. An early study demonstrated that synaptic decline is likely the result of axonal dysfunction affecting the presynaptic termini. Gene expression studies in AD validate these findings, as proteins involved in synaptic vesicle trafficking and neurotransmitter recycling, in addition to structural elements of the synapse, are affected. These changes appear to be directly related to AD pathology, and their effects on synaptic function are associated with clinical symptoms. The route of synaptic damage may be multifocal, as impaired axonal transport resulting from tau dysfunction may be complemented by the arrival of hyperphosphorylated tau in dendritic spines and concomitant impaired synaptic transmission.
Injection of Aβ fibrils into the brains of non-human primates and of P301L tau transgenic mice induced tau hyperphosphorylation and NFT formation. Also, hyperphosphorylation of tau was induced by an aqueous extract containing soluble Aβ oligomers from AD brains, but not by an extract from non-AD brains. Additionally, several studies showed that Aβ fibrils induced tau hyperphosphorylation in vitro and that Aβ fibrils in tau KO mice did not cause hippocampal neuron degeneration, suggesting that tau and its phosphorylation state is one of the major pathological downstream targets of Aβ oligomers. Indeed, in cultured mature hippocampal neurons and in neuroblastoma cells, soluble Aβ oligomers stimulated tau phosphorylation at epitopes characteristically hyperphosphorylated in AD, namely Ser404, Thr231, Thr181, Ser202 and Thr205. And, finally, a monoclonal antibody that targets soluble Aβ oligomers blocked Aβ attachment to synaptic binding sites and prevented tau hyperphosphorylation. Thus, the properties of soluble Aβ oligomers and of hyperphosphorylated tau appear capable of accounting for key features of AD pathology and cognitive failure, consistent with the idea that soluble Aβ oligomers trigger tau hyperphosphorylation and aggregation in NFTs.
In 1991 Hardy and coworkers proposed the influential amyloid cascade hypothesis (ACH) that placed Aβ peptides at the center of AD pathogenesis. The idea was that Aβ — either in fibrillar plaques or as soluble oligomers — triggers a pathophysiological cascade leading to tau misfolding and assembly that spreads throughout the cortex, ultimately resulting in neural system failure, neurodegeneration and cognitive decline. This ‘linear’ pathogenesis model spawned an avalanche of AD clinical trials with drugs targeting different aspects of Aβ metabolism: β- and γ-secretase inhibitors (to inhibit Aβ production), aggregation inhibitors (to limit/reverse Aβ aggregation), protease regulators and Aβ-specific antibodies (to clear Aβ monomers, soluble oligomers, fibrils and aggregates). However, beginning in 2003 reports of trial failures or abandonments began to appear. Currently, no new effective drug has emerged from the trials whose list of failures numbers over 200, constituting a major blow to the ACH. Ongoing follow-up clinical trials, deploying cutting edge technological advancements, aim to address supposed design failures, including (1) late initiation of therapeutic interventions, (2) inappropriate drug dosages and (3) inappropriate selection of trial participants.
Evidence from multiple models and experimental settings now provides strong support for Aβ-tau synergy throughout the disease course, rather than sequentially as proposed by the ACH. For example, in experiments with APP/PS1 transgenic mice and with double mutant APP/tau transgenic mice, tau pathology exacerbated Aβ deposition. A synergistic association between Aβ and tau predicting longitudinal memory decline was also demonstrated in a CSF biomarker study of individuals aged 50–90 years, in which total tau and phosphorylated-tau (p-tau) levels correlated with cognitive performance only when Aβ deposition (as evidenced by low CSF Aβ) was contemporaneously present. In cell culture, the addition of Aβ to human cells expressing (wild-type) tau resulted, after a 5-day period, in tau aggregate formation in the form of PHFs, the major components of tangles. Cortical and/or hippocampal Aβ injections (both synthetic and brain-derived) into P301L-mutant tau mice accelerated tangle formation, not only near the injection site but also in synaptically connected areas. Similarly, injection of human brain-derived PHFs into APP/PS1 mice (5xFAD model) enhanced cortical tau propagation compared to injections into wild-type mice. Notably, PHF injection exacerbated tau pathology particularly near plaques. This latter aspect was further investigated in another study, in which hippocampal injections of tau seeds derived from AD-brain homogenates into APPNL-F-knock-in and 5xFAD mice induced tau aggregation, especially in plaque-associated dystrophic neurites, leading to the development of ‘neuritic’ plaques (i.e., Aβ deposits with associated neurofibrillary tau-dystrophic neurites). This finding was independently confirmed, reinforcing the idea that plaques provide a microenvironment that promotes tau aggregation and propagation and hence disease progression.
These finding could not only help explain the negative results from anti-Aβ clinical trials but also suggests that clinical trials directed solely at tau may also need to be reconsidered. Few studies have examined the role of anti-Aβ therapy in the context of Aβxtau models, but results are consistent with the idea of Aβ-dependent and independent phases of pathogenesis. In the 3xTg model, that overexpresses both APP and tau, antibodies directed against Aβ reduced tau pathology at early but not later stages of the disease when tau becomes hyperphosphorylated and resistant to clearance. Anti-oligomeric Aβ antibodies were effective in reducing plaques, tau hyperphosphorylation and microglia activation, and they improved cognition in the same mouse line — a likely prophylactic effect taking place before substantial tau accumulation. Similarly, early active immunization against Aβ also prevented Aβ and tau accumulation in the 3xTg model. Whether anti-Aβ treatment alone can rescue neuronal dysfunction in mice that have established plaque and tangle pathology has not been tested, but is unlikely, given that the combination of Aβ and tau leads to a phenotype that is different than Aβ alone and that is dominated by tau-dependent neuronal silencing. Remarkably, tau suppression was incapable of rescuing tau-dependent neuronal silencing in two APPxtau models (at least during the 6 weeks of applied treatment) and was unable to reduce the number of tau-positive neurons in 12-month-old APP/PS1-rTg4510 animals to the level seen in treated rTg4510 mice, despite similar decreases in levels of soluble tau. These studies reinforce the notion of Aβ-tau synergy and indicate that combined anti-Aβ and anti-tau therapies may be the most effective way to improve neuronal function (by reducing Aβ effects and by decreasing tau formation and increasing its rate of clearance). Moreover, since tau is more stable in the presence of Aβ, with both a presumed longer half-life and increased bioactivity, a combinatorial therapeutic approach implemented at early stages of the disease would exploit the inverse relationship between rate of tau turnover and presence of Aβ, and at the early stage, therapeutic tau clearance would be reciprocally enhanced by concurrent anti-Aβ therapy. Therapeutic development in AD has been rooted in the notion that Aβ and tau are co-existing species that are temporally, but not synergistically, related. However, compelling experimental and clinical data now support a co-pathogenic interaction between Aβ and tau in AD, which not only manifests throughout the disease course, but also fundamentally drives disease progression.
(adapted from: Walton, C., Begelman, D., Nguyen, W., Andersen, J. (2020). Senescence as an Amyloid Cascade: The Amyloid Senescence Hypothesis Frontiers in Cellular Neuroscience 14(), 129; McAllister, B., Lacoursiere, S., Sutherland, R., Mohajerani, M. (2020). Intracerebral seeding of amyloid-β and tau pathology in mice: factors underlying prion-like spreading and comparisons with α-synuclein Neuroscience & Biobehavioral Reviews 112(), 1-27; Abramov, A., Potapova, E., Dremin, V., Dunaev, A. (2020). Interaction of Oxidative Stress and Misfolded Proteins in the Mechanism of Neurodegeneration Life 10(7), 101; Ricciarelli, R., Fedele, E. (2017). The Amyloid Cascade Hypothesis in Alzheimer’s Disease: It’s Time to Change Our Mind Current Neuropharmacology 15(6); Chávez-Gutiérrez, L., Szaruga, M. (2020). Mechanisms of neurodegeneration — Insights from familial Alzheimer’s disease Seminars in Cell & Developmental Biology; Felice, F., Wu, D., Lambert, M., Fernandez, S., Velasco, P., Lacor, P., Bigio, E., Jerecic, J., Acton, P., Shughrue, P., Chen-Dodson, E., Kinney, G., Klein, W. (2008). Alzheimer's disease-type neuronal tau hyperphosphorylation induced by Aβ oligomers Neurobiology of Aging 29(9), 1334-1347; DeTure, M., Dickson, D. (2019). The neuropathological diagnosis of Alzheimer’s disease Molecular Neurodegeneration 14(1), 32; Berchtold, N., Cotman, C. (1998). Evolution in the Conceptualization of Dementia and Alzheimer’s Disease: Greco-Roman Period to the 1960s Neurobiology of Aging 19(3), 173-189; Hillen, H. (2019). The Beta Amyloid Dysfunction (BAD) Hypothesis for Alzheimer’s Disease Frontiers in Neuroscience 13(), 1154; Lauritzen, I., Pardossi-Piquard, R., Bauer, C., Brigham, E., Abraham, J., Ranaldi, S., Fraser, P., St-George-Hyslop, P., Thuc, O., Espin, V., Chami, L., Dunys, J., Checler, F. (2012). The β-Secretase-Derived C-Terminal Fragment of βAPP, C99, But Not Aβ, Is a Key Contributor to Early Intraneuronal Lesions in Triple-Transgenic Mouse Hippocampus The Journal of Neuroscience 32(46), 16243-16255; LaFerla, F., Green, K., Oddo, S. (2007). Intracellular amyloid-β in Alzheimer's disease Nature Reviews Neuroscience 8(7), 499-509; Oddo, S., Caccamo, A., Shepherd, J., Murphy, M., Golde, T., Kayed, R., Metherate, R., Mattson, M., Akbari, Y., LaFerla, F. (2003). Triple-Transgenic Model of Alzheimer's Disease with Plaques and Tangles Intracellular Aβ and Synaptic Dysfunction Neuron 39(3), 409-421; Busche, M., Hyman, B. (2020). Synergy between amyloid-β and tau in Alzheimer’s disease Nature Neuroscience 23(10), 1183-1193; and Yipeng Wang, Eckhard Mandelkow (2016) Tau in physiology and pathology Nature Reviews Neuroscience)